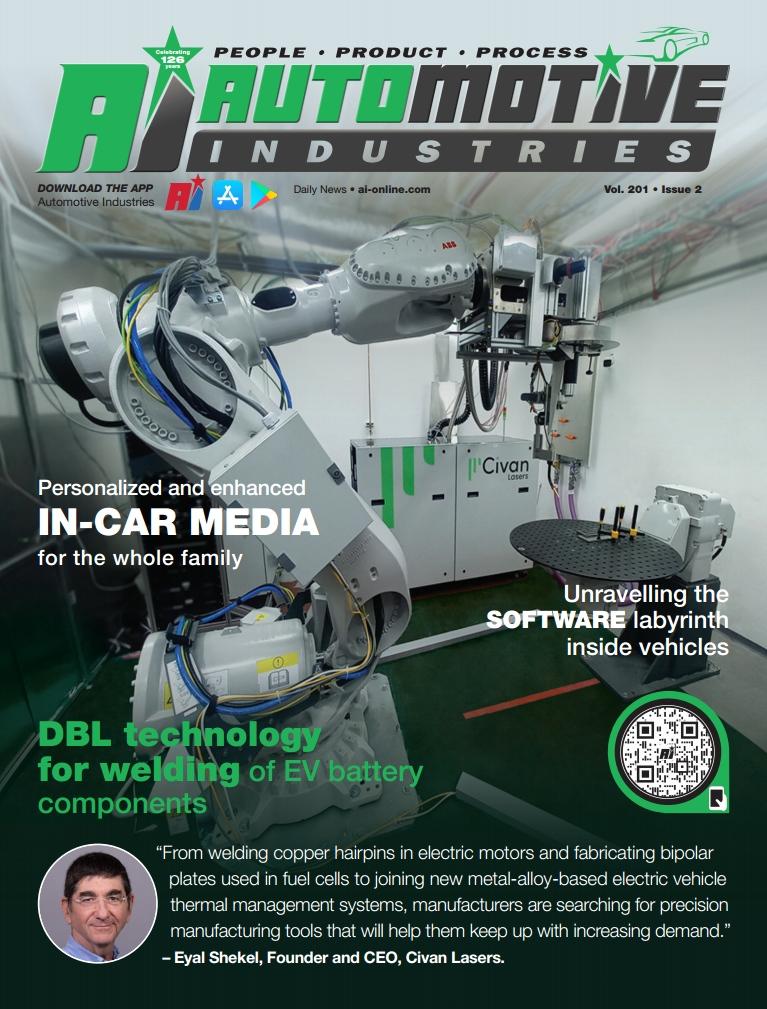
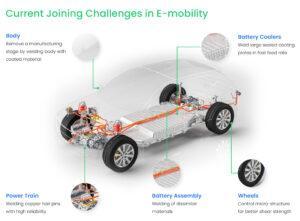
The quest for e-mobility presents challenges that automotive original equipment manufacturers (OEMs) don’t have to contend with during production of vehicles with internal combustion engines (ICEs). For example, materials used to produce electric vehicle (EVs) can be challenging. These include low-absorption materials such as copper and aluminum, materials with low viscosity such as aluminum, and aluminum alloy and brass elements with low boiling temperatures.
EVs also require a whole new class of components that are larger in scale and have more complex geometries than ICE vehicle components. Finally, EVs require improved process efficiency and speed because certain components may require hundreds of joints.
From welding copper hairpins in electric motors and fabricating bipolar plates used in fuel cells to joining new metal-alloy-based electric vehicle thermal management systems, manufacturers are searching for precision manufacturing tools that will help them keep up with increasing demand (Figure 1). These new materials processing tools need to weld, cut, combine, and process new products with advanced specifications while keeping up with production throughput as part of an overall cost-effective solution.
Manufacturers seeking the best solutions are increasingly switching from traditional mechanical machining and thermal welding applications to technologies that involve the use of new materials, reduce power consumption, and achieve better process yields. One such technology is laser materials processing (Figure 2).
Enter Laser Welding
Widespread availability of multi-kilowatt lasers with increased reliability and robustness has accelerated the adoption of laser materials processing technology. Compared to the traditional thermal, stick, MIG, and TIG welding processes used for decades in joining and welding operations in the production of ICE vehicles, laser welding requires less maintenance, reduces production costs, and increases manufacturing flexibility and selectivity.
Another benefit of laser welding technology is that it requires less heat input due to the high energy of the beam, which minimizes the potential for distortion while maximizing speed. While traditional ICE vehicle applications have been well served by old-school thermal welding technologies, these mature technologies have been unable to meet the challenges presented by e-mobility.
While all welding methods involve melt pool formation and subsequent rapid solidification, which alters the properties and microstructure of the welded metal, the high energy of laser welding not only melts material but also evaporates it.1 This evaporation of material during the welding process creates a vapor capillary, also called a vapor keyhole. This keyhole gives laser welding the advantage of a very high aspect ratio, or a high ratio of penetration depth to weld seam width (Figure 3).
The Keyhole Stability Challenge
Compared to thermal welding methods, which generally provide wider and shallower weld seams, laser welding’s high aspect ratio also translates into lower part distortion potential. While the keyhole gives laser welding the aforementioned advantages over thermal welding, it can also pose challenges, since keyhole stability is crucial for achieving high weld quality.
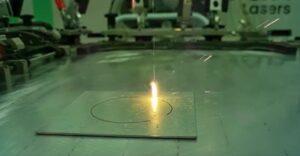
During the welding of high-absorption materials such as steel and nickel, the keyhole generally remains stable, enabling laser welding to achieve higher processing speeds, less distortion, and better weld quality. Unfortunately, during the welding of copper, aluminum, and high alloy materials, which is commonly required in e-mobility applications, the keyhole can be inherently unstable, making the process susceptible to irregularities that compromise weld quality, such as pores, spatter (melt ejection), and humping.
Despite these challenges, automotive manufacturers working to electrify their products increasingly turn to new laser-based materials processing. They keep up with production throughput by tailoring process parameters for each application to maintain keyhole stability and weld quality.
In laser welding, for example, manufacturers can tailor process parameters such as wavelength, pulse energy, pulse duration, repetition rate, pulse overlap, motion speed, and intensity profile, as well as laser beam spot shape and size, for each application. Industry currently uses several different approaches, including wobble welding with a fast scanner, laser sources with a visible green or blue wavelength, and variable superimposed intensity distribution with a two-in-one (dual-core) fiber.2
Welding Copper Hairpins
The beneficial electromagnetic and thermal performance of hairpin winding technology in electric motors helps manufacturers meet increasing power density and efficiency requirements. This is especially important for electric motors used in hybrid EV (HEV), plug-in HEV, full EV, and fuel cell vehicle power trains. By replacing traditional stranded wire with solid conductors, hairpin windings achieve a higher fill factor and improved thermal performance and help make highly automated manufacturing possible.
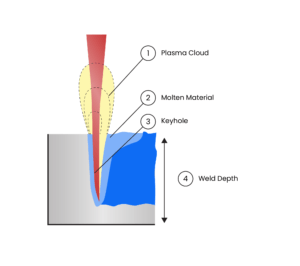
During such automated production, processes generally include slot insulation; hairpin forming and insertion; and enlargement, twisting, cutting, repositioning, and laser welding of hairpin terminal wires.3 To deliver good electrical properties, hairpin welding requires high laser power and welding speed with minimal spatter and pore defects.
Due to its high electrical and thermal conductivity, hairpins are typically made of copper. When welding copper, its high thermal conductivity presents one of the biggest challenges. The heat conduction of copper is 10 times higher than that of commonly welded steels. So copper requires very intense energy input. While laser welding is generally a good fit for addressing these needs, another challenge is copper’s high reflectivity; copper and other reflective metals typically absorb only a small fraction of the near infrared (near IR) spectrum laser light employed by most welding lasers.
As a result, only about 5% of the near IR laser energy can be used for heating up material in copper welding.4 Heating copper from room temperature, where absorption of the laser light is low to initiate the welding process, requires high intensity because the surface of copper at room temperature reflects most of the laser power to the surroundings. This inefficiency improves as IR light absorption increases with temperature, up to more than 15% at melting temperature, but this transition is hard to control.5
Green Light Absorbs Better
Compared to steel, copper’s decreased surface tension and viscosity make the melt pool less stable. High intensity at start, combined with increasing absorption, means the material could overheat and makes heat conduction welding difficult to reliably reproduce. In contrast, deep-penetration welding suffers from weld defects such as spatter, especially at a slower feed rates.6
Absorption of green laser light (515–537 nm) in copper is 35–40%. As a result, the stability of the welding process can be significantly improved by eliminating problems associated with initial absorption and overheating. Green lasers make it possible to reproduce high-quality welds in small copper parts up to 0.4 mm thick and even foils. The greater absorption of green laser light in copper improves keyhole and melt pool dynamics to help spatter-free copper welds be more reproducible.7
While conventional green lasers can achieve 100 mm/sec feed rates with copper hairpin welding, yields are low due to penetration depths of less than 1 mm. Without deep penetration welding of copper, it’s difficult to achieve highly reliable bonds with low electrical resistance and increased mechanical strength to optimize durability and life span. DBLs can achieve the same 100 mm/sec feed rate, at greater than 1 mm penetration de
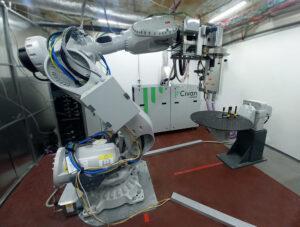
pths, resulting in higher-quality welds and higher yields, reducing waste.
Possible Fuel Cell Solutions
Fuel cell–powered EVs require a fuel cell stack comprising bipolar plates — thin plates of just hundreds of microns. Each cell contains 300 to 400 plates with a weld seam of 3 to 6 meters. Currently, it takes 10 times longer to weld the plates than to complete the rest of the manufacturing process (Figure 4).
As welding is the critical path to production, cutting this time has a significant effect on production time and the environmental impact of the production process. While there are many efforts to increase welding speed to keep up with demand, increasing the feed rate to more than 0.5 m/sec results in welding defects caused by the well-known humping effect, leading to faulty parts and a backlog of materials.
For a bipolar plate to be welded properly, it must pass a hydrogen leakage test, ensuring that the weld is tight and there are no leaks. While a plate may pass the leak test even with humping, humping can change the shape enough to prevent plates from stacking neatly into the space in the vehicle allotted for the fuel cell stack (Figure 5). Humping increases at high speeds with high-power lasers because control of the keyhole and melt pool decreases.
While the shape of standard laser beams cannot be easily changed, especially mid-process, according to recent findings from the Eureka Project, based at Fraunhofer Labs in Aachen, Germany, DBL technology, which enables real-time control of beam shape to decrease the risk of humping, may provide the automotive industry with a technological solution for economically mass-producing clean-energy engines through
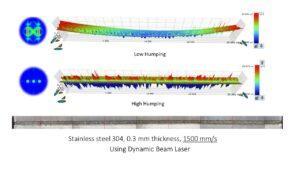
an increased feed rate for bipolar plate welding.
Welding EV Battery Cooling Plates
Another challenge for OEMs replacing combustion engines with electric motors and large battery packs is the production of battery coolers, critical components in battery thermal management systems. EV battery cooling plates regulate the temperature of the battery pack and some of the electronics by circulating coolant through channels in a welded base plate.
Welding of battery cooling plates requires very high-quality hermetically sealed joints to prevent leakage of the circulating coolant. The joints cannot have any cracks because they lead to mechanical failure. With the adoption of new metal-alloy-based cooling system designs, manufacturers are searching for faster, more efficient joining methods that will help them keep up with demand.
Today’s EV battery systems require cooling plates measuring about 2.1 x 1.3 meters (Figure 6). The larger cooling plates, combined with new materials that offer improved mechanical properties and recyclability, such as 5xxx and 6xxx Al alloys, push the limits of modern joining technologies. Currently, manufacturers use vacuum brazing technology.
Brazing is inefficient, takes up a lot of space, and is slow. The brazing process also requires the use of Al 3003, a special aluminum alloy that can be brazed. Manufacturers want to switch to a more economical alloy called Al 5754, which cannot be brazed.
Feed Rate Limitations
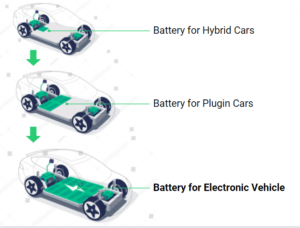
Conventional fiber lasers cannot achieve the high feed rates required because such welding speeds result in cracks, porosity, and humping, which ruin joint integrity (Figure 7). The maximum feed rate of traditional lasers for welding Al 3003 is 8–12 m/min. The rate is less than 15 m/min for Al 5754.
Due to feed rate limitations, such laser welding methods are not viable solutions to meet increasing demand because manufacturers must purchase and operate multiple laser welding stations to overcome the throughput bottleneck, which significantly increases capital equipment costs and energy consumption.
DBLs overcome feed rate limitations when welding cooling plates for EV battery thermal management systems. Thanks to dynamic beam shaping, shape frequency of up to 50 MHz, shape sequences, and focus steering, DBLs achieve feed rates of 30 m/min, which is more than two times faster than brazing.
DBL software makes it easy to design the relevant beam shape, frequency, sequence, and focus; upload it to the laser; and see the effect on the weld using cross-section analysis. The simplicity and speed of this process make it possible to evaluate multiple shapes and optimize the best shape for welding the cooling plates (Figure 8).
The Business Case
DBL technology, which allows manufacturers to control beam shape, frequency, sequence, and focus steering, can improve weld quality and processing speed when welding cooling plates. Because DBL
technology allows precise control of beam shape, sequence, frequency, and focal depth, the laser can be easily optimized to meet specific welding requirements and can cost-effectively join aluminum alloy plates at feed rates of 30 m/min without defects.
DBL technology offers various solutions for disparate cooling plate designs made from Al 3003, Al 3103, and Al 5754 alloys. In these materials, DBL technology can achieve stable welding of cooling plate se
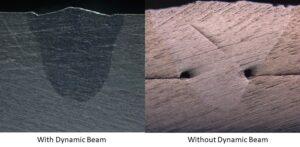
ams at feed rates of 30 m/min. The DBL can create 30 meter–long weld seams with consistent geometry on each plate. While the advantages noted above can be leveraged in many ways across a wide range of materials processing applications, testing has revealed that cooling plates welded with DBL technology meet customer requirements in metallographic, mechanical, and leak tests.
DBL technology not only opens new possibilities for laser welding but also allows tier 1 automotive companies to replace inefficient joining methods and to achieve a competitive edge when manufacturing cooling plates with strong, leak-free welds.
Conclusion
The advantages noted above can be leveraged in many ways across a wide range of materials processing applications. Moving the beam and focus at speeds of hundreds of megahertz traces various beam shape patterns to distribute energy over the work surface as needed to tailor the beam for a variety of other applications, including welding dissimilar materials with different reflectivity, heat capacity, and melting points and joining crack-sensitive materials.
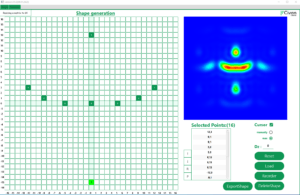
The DBL platform makes it easy to quickly test different beam shapes and frequencies to define the optimal process for high-quality welds. Users can conduct a multitude of tests in a brief period of time and quickly see results. By changing one condition at a time, users can easily identify the ideal shape, frequency, and feed rate for the strongest weld, with minimal spatter and porosity, regardless of the application.
References
- Behzad Fotovvati, Steven F. Wayne, Gladius Lewis, and Ebrahim Asadi, “A Review on Melt-Pool Characteristics in Laser Welding of Metals,” Advances in Materials Science and Engineering (2018), https://doi.org/10.1155/2018/4920718.
- Eric Punzel, Florian Hugger, Robert Dörringer, Thorm Lembit Dinkelbah, and Andreas Bürger, “Comparison of Different System Technologies for Continuous-Wave Laser Beam Welding of Copper,” Procedia CIRP 94 (2020):587–91, https://doi.org/10.1016/j.procir.2020.09.081.
- “Hairpin Technology for Automated Assembling,” Electric Motor Engineering, November 18, 2017, https://www.electricmotorengineering.com/__trashed.
- Bliedtner, H. Müller, and A. Barz, Lasermaterialbearbeitung Grundlagen – Verfahren –Anwendungen – Beispiele, Hanser, Munich, Germany (2013). (“Material loss analysis in glass additive manufacturing by laser glass …”)
- Heider, J. Sollinger, F. Abt, M. Boley, R. Weber, and T. Graf, “High-Speed X-Ray Analysis of Spatter Formation in Laser Welding of Copper,” Physics Procedia 41 (2013):112–18.
- Engler, R. Ramsayser, and R. Proprame, Physics Procedia 12 (2011):342–49.
- Henrikki Pantsar, Eva-Maria Dold, Marc Kirchhoff, and Oliver Bocksrocker, “Last Welding at the Green Wavelength Benefits Electrified Mobility Applications,” Laser Focus World, December 31, 2018, https://www.laserfocusworld.com/industrial-laser-solutions/article/14215476/laser-welding-at-the-green-wavelength-benefits-electrified-mobility-applications.
More Stories
Is Your 70 Series Land Cruiser Underperforming? Here’s How to Unleash Its True Potential
What to Do After a Motorcycle Accident to Protect Yourself
Injured in a Motorcycle Crash? Talk to an Attorney Now